Anchoring students’ activities with a narrative helps them to situate what they are doing in a larger problem space. In observations of EiE classrooms, we often see students evoke the storybook character or the setting as they work on their solutions. Here’s an example from Ms. Smith’s second-grade class, where students are working on the EiE unit A Stick in the Mud: Evaluating a Landscape (EiE, 2011 http://www.eie.org/eie-curriculum/curriculum-units/stick-mud-evaluating-landscape). They must choose a site for a TarPul, a kind of gondola used to cross rivers (see Figure 1). To set a context for the design challenge, they have read the EiE storybook Suman Crosses the Karnali River, about a boy who lives in a village in Nepal that is getting a new TarPul. Suman worries about where the TarPul will be sited because his grandmother is ill and sometimes needs to cross the river to the nearest clinic; he wants her trip to the clinic to be short and easy. Each group of students creates a model of the type of soil (rocky, organic, or both) and its compaction present at the site they selected and then tests how much weight a model TarPul bridge will support. Figure 2 shows a testing set-up. In the transcript below, as they weigh the criteria for the optimal placement of a Tarpul bridge, the students also draw upon the storybook scenario to generate a solution. The grandmother is sick and needs to travel to the clinic; the teacher helps the students to realize that a safe design calls for a gondola that can carry at least two people (more than that would also be fine).
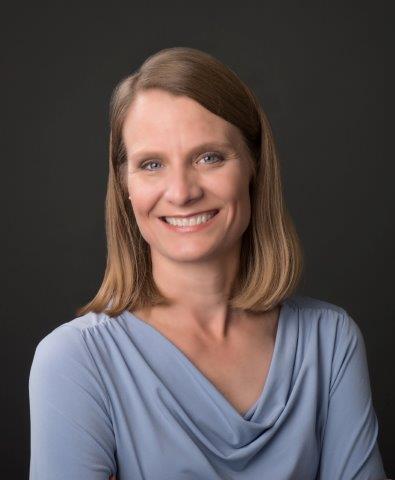
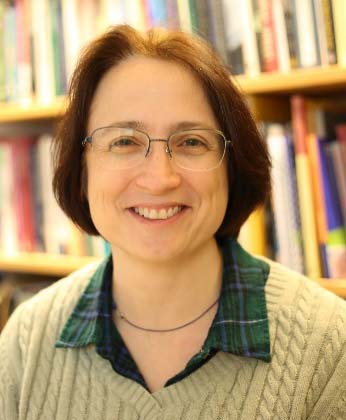
Designing Engineering Experiences to Engage All Students
Christine M. Cunningham and Cathy P. Lachapelle
Museum of Science, Boston
Abstract
iNew academic standards at the state and national level in the U.S. A. call for integrating engineering into K–12 education. As designers develop curricula to meet this new need, we must ensure that engineering instruction is inviting and engaging for all students, particularly those from populations that are underserved, underperforming, or underrepresented in STEM fields. Starting with the explicit goal of fostering equity, we designed an engineering curriculum for grades 1 - 5. In this paper we articulate the set of 14 inclusive design principles that guided the development of the Engineering is Elementary (EiE) curriculum, link them to relevant literature, provide examples of how they influenced our design decisions, and describe classroom outcomes.
Introduction
iiThe recent introduction of a new discipline – engineering – into K-12 education in the U.S.A. comes with both opportunities and responsibilities. One particular opportunity for curriculum designers stems from the reality that few entrenched models of K-12 engineering instruction exist, so we can start fresh, with an eye toward best practices. At the same time, we have a responsibility to promote educational equity and work diligently toward closing the achievement gap (also called the opportunity gap); colleges, universities, and workplaces in the U.S.A. have an unfortunate (though unintended) track record of excluding certain groups from engineering instruction and employment (Burke & Mattis, 2007) [1].
Little research has been done on young children’s interest in engineering. But research on children’s interest in, and attitudes toward science can inform the design of an engineering curriculum because of the many similarities in concepts and skills used in engineering and science. Science education researchers conclude that it is important to address disparities in access and exposure to science at an early age because students’ interest in science tends to decline after elementary school (Brotman & Moore, 2008; Catsambis, 1995; Clewell & Braddock, 2000; Reid & Skryabina, 2003). From the earliest school years, girls’ attitudes toward science tend to be more negative than those of boys, and both boys and girls tend to show increasingly negative attitudes as they get older, with girls’ attitudes becoming disproportionately more negative than boys’ (Catsambis, 1995; Reid & Skryabina, 2003). One exception to this pattern is African American girls, who tend to have more positive attitudes toward science than both African American boys and white girls (Hanson, 2009). Students from cultures and groups underrepresented in science, such as English-language learners and students from high-poverty areas, may have more trouble in navigating the differences between their home cultures and the culture of science than do students from well-represented groups. This can lead to difficulties with achievement even where interest exists (Aikenhead & Jegede, 1999; Lee, 2003).
Engineering is Elementary
iiiIn 2003 our team at the Museum of Science, Boston began to develop Engineering is Elementary (EiE)®, a curriculum that introduces elementary school-aged children to principles of engineering and technology. Over the past 13 years, EiE curricular units have been used in classrooms across the country; as of February 2016 approximately 10 million children and 110,000 teachers have used our materials. The impact of EiE has far exceeded our initial expectations. Research and evaluation data suggest that EiE materials are engaging for girls, children of color, children from low socioeconomic groups, and children with disabilities and have resulted in learning gains related to both engineering and science (Lachapelle, Cunningham, Jocz, Kay, Lee-St. John et al., 2011; Lachapelle, Cunningham, Jocz, Kay, Phadnis et al., 2011, Weis & Banilower, 2010). We believe these results can be attributed, in part, to the commitments we brought to our work.
Grounding our efforts was the belief in the benefits to individuals, engineering disciplines, and society when people engage in problem solving, innovation, inquiry, and engineering design. We resolved to design materials that would effectively reach all children, attracting and engaging underrepresented, underperforming, and underserved students, including girls, minorities in STEM, students from low socioeconomic backgrounds, students with individualized education plans, and English-language learners. From the beginning, we worked to identify design principles that would be maximally inclusive; in pilot tests and evaluations, we collected data on student demographics so we could examine the effects of the curriculum on different populations.
Briefly, we concluded that an equitable, inclusive engineering curriculum must demonstrate the relevance of engineering in the real world. It must actively engage students in engineering practices as they work on developmentally appropriate design challenges. It must include scaffolding to support students as they learn key concepts and practices and become fluent with the cultural norms of engineering. It must engender learning environments where students can contribute, collaborate, and develop their own sense of agency, expertise, and ownership. These criteria are similar to what others have advocated for science education (Brotman & Moore, 2008; Carlone, Haun-Frank, & Webb, 2011); however, engineering education affords unique opportunities heretofore unrecognized.
We also argue that project-based learning sets the stage for equitable instruction in engineering. Like educators who advocate for project-based learning in science (Krajcik & Blumenfeld, 2006), we view this approach as contributing to student motivation and engagement, to deeper learning of concepts, and to a broader learning of the practices, discourses, and culture of the discipline. The five key criteria for project-based learning are that students should (1) start with a problem they are challenged to solve; (2) explore the dimensions of the problem and possible solutions using guided inquiry; (3) work collaboratively to develop designs to solve the problem; (4) have their performance scaffolded so they can engage in new practices and participate at a deeper level than if left to their own devices; and (5) produce designed, iteratively improved solutions and share them with the classroom community or others (Blumenfeld, Soloway, Marx, Krajcik, Guzdial, & Palincsar, 1991). We applied these criteria to the design of the EiE curriculum.
With equity as the focus, drawing on educational literature and our experiences working in classrooms, we developed a set of 14 design principles for curricula and materials that guided the development of EiE. These principles can be grouped in four larger categories as illustrated by Table 1.
Category |
Design Principles |
---|---|
Set learning in a real world context |
Use narratives to develop and motivate students’ understanding of engineering’s place in the world. |
Demonstrate how engineers help people, animals, the environment, or society. |
|
Provide role models with a range of demographic characteristics. |
|
Present design challenges that are authentic to engineering practice |
Ensure that design challenges are truly open-ended, with more than one ‘correct’ answer. |
Value failure for what it teaches. |
|
Produce design challenges that can be evaluated with both qualitative and quantitative measures. |
|
Cultivate collaboration and teamwork. |
|
Engage students in active, hands-on, inquiry-based engineering. |
|
Scaffold student work |
Model and make explicit the practices of engineering. |
Assume no previous familiarity with materials, tasks, or terminology. |
|
Produce activities and lessons that are flexible to the needs and abilities of different kinds of learners. |
|
Demonstrate that “everyone can engineer” |
Cultivate learning environments in which all students’ ideas and contributions have value. |
Foster children’s agency as engineers. |
|
Develop challenges that require low-cost, readily available materials. |
In the sections that follow, we describe these principles, link them to relevant literature, and show how they have influenced design decisions and classroom outcomes. The 14 EiE design principles are offered not as a comprehensive list but rather as a starting place that should be continually discussed, researched, and revised.
Inclusive Curriculum Design Principles
ivCategory 1: Set learning in a real-world context
Research on K-12 science education finds “many students who are academically competent in the school subject matter ultimately view school’s knowledge and skills as irrelevant for their future career and/or everyday lives” (Carlone et al., 2011, p. 2). To promote student interest in engineering and science as fields of study and as future career opportunities, educators should help students see how the topics they are learning are relevant in the real world. Students also need to be able to picture themselves filling such roles in the future. This is especially important for students from traditionally underrepresented populations.
Putting learning in a real-world context increases students’ engagement, enthusiasm, and achievement (Kang & Lundeberg, 2010; Lieberman & Hoody, 1998). Emphasizing the social and societal connections – the place of engineering and science in the development of knowledge and technology, the role of engineers and scientists in driving theory-building and technological change, and the effects of science and engineering on all aspects of modern life – increases student interest and motivates students to drive their own learning and achievement (Brotman & Moore, 2008). The three design principles that set a real-world context for all students, but particularly groups underrepresented in engineering, are described below.
Principle 1: Use narratives to develop and motivate students’ understanding of engineering’s place in the world
Narrative is humanity’s natural means of learning and remembering (Wilson, 2002). Students connect to stories at a personal level (Stinner, 1996). A coherent narrative is a natural point of entry into the culture and aesthetic of a new discipline such as engineering (Martin & Brouwer, 1991) and engages students in constructing their own understanding.
“So what?” is a perennial question asked by students in schools, particularly when science instruction is divorced from direct experience (Buxton, 2010). Providing a compelling narrative or context benefits all students, but it can particularly boost the participation of girls and other underrepresented students who may be less predisposed to identify with or apply themselves to more technical studies or the physical sciences (Bennett, Lubben & Hogarth, 2007; Casey, Erkut, Ceder & Young, 2008; Halpern et al, 2007).
Narrative stories and controversies that address science and engineering as they apply to people’s lives have the potential to powerfully affect students’ attitudes toward and understanding of science, engineering, and technology and the ways in which they are practiced (Koul & Dana, 1997). Narratives help raise questions in students’ minds, arousing their curiosity and imagination (Klassen, 2009). Connecting school learning to events in the real world can motivate students by providing relevance for what they are learning, whether that is science or technological problems and problem solving (Baker & Leary, 1995; Buxton, 2010; Klassen, 2007).
To help students situate their tasks in the real world, each EiE unit begins with a short work of fiction written expressly for that unit – a storybook introducing a problem that is the same as (or similar to) the one students will encounter in class. In the storybook, a child protagonist solves the problem with some advice from an adult mentor.
Ms. S:Can you tell me how many weights you got [into the model TarPul]? … If I said to you, 10 of those cubes equals one person, how many people could you put in your TarPul to cross the river? Sam?
Sam:26.
Ms. S:How many people would that be?
Sam:Two. Two and a little bit.
Ms. S:Let’s be safe and call that two. We won’t count the leftovers. Ellie? How many weights could you put in?
Ellie:36. That’s like three people.
Ms. S:How about the rest of you? Jenny?
Jenny:We got 44 weights in. That’s, um, 4 people.
PS:Jessica?
Jessica:We got 15 weights in the cup. (Ms. S: prompts) One person.
Observer:What would be the safest number?
Alex:Two people.
Ms. S:Why would two be the safest?
Ellie:Because if you were with your family, you might need to be with someone else.
Jenny:If someone can’t move, they can’t pull themselves across.
Ellie:And if you’re a little kid with your mother, or if you’re sick, you need someone to help you get across.
Principle 2: Demonstrate how engineers help people, animals, the environment, or society
Research finds that many students, but particularly girls and underrepresented minorities, are interested in ‘helping careers’ (Baker & Leary, 1995; Jones, Howe, & Rua, 2000; Miller, Blessing, & Schwartz, 2006). Girls want to see how school subjects are relevant to their lives, or to see social value (Burke, 2007); their attitudes toward school subjects are affected by these perceptions of relevance (Clewell & Braddock, 2000). Looking at college-bound high school students, Miller, Blessing, and Schwartz (2006) found that girls tended to choose people-oriented majors; when choosing a science or engineering major, they tended to focus on fields like biology, which they saw as allowing them to help animals or people, particularly through health professions. Meanwhile students of both genders hold stereotypical views about physical science being for boys and biological science being for girls (Jones et al., 2000); girls prefer and choose to participate more frequently in biological sciences than in physical sciences and engineering (Buccheri, Gurber, & Bruhwiler, 2011; Dawson, 2000; Drechsel, Carstensen, & Prenzel, 2011; Miller et al., 2006).
At the grade school level, however, engineering activities from other curricula overwhelmingly focus on the physical sciences (typical examples include designing robots, model race cars, or potato launchers) with no obvious connection to helping professions. EiE units are specifically designed to make the ‘helping’ aspect and positive societal impacts of engineering transparent, through both the storybook and the core design challenge. For example, one EiE unit focuses on electrical engineering, a field that has not traditionally attracted large numbers of women. Instead of presenting circuit construction as a decontextualized exercise, the unit engages students in designing circuits as one component of a larger alarm system that ensures baby lambs have enough water in their drinking trough by alerting the caregiver when the water level gets low. A motivational context that helps students understand how their efforts benefit someone (in this case both the caregiver and the lambs) can be very engaging for children.
Principle 3: Provide role models with a range of demographic characteristics
Girls perceive science as difficult, uninteresting, and resulting in an unattractive lifestyle (Burke, 2007; Miller et al., 2006). In part that is because they receive mixed messages about careers and gender roles from the media; however, presenting a variety of positive role models – both sexes, varied races and ethnicities, different abilities/disabilities, a wide range of hobbies and interests – can combat these perceptions (Baker & Leary, 1995).
The 20 EiE units were intentionally planned to highlight a diverse group of people engaging in engineering; early in the design process, we created a chart of attributes and assigned them to the storybook protagonists and their mentors with the goal that every student could find at least one character to identify with. The storybook children are male and female, enjoy a variety of hobbies, have varied personalities, and live in different family structures. Some have disabilities: there’s a child who uses a wheelchair, a child who is blind, a child with Down Syndrome, and a child with a limb birth defect. And the stories are set in countries around the globe, so the protagonists represent a variety of races and ethnicities. They truly illustrate that everyone can be an engineer.
This design choice makes a difference. In EiE classrooms, it’s common to hear a child exclaim, “That [storybook character] looks like me!” or that a certain protagonist is his or her favorite. We hear from teachers that immigrant children became stars after the class reads a story set in their birth country – they teach their classmates more about their native language or customs. One teacher reported that her class had a long and sensitive discussion about Down Syndrome after students learned during whole-class reading time that one child in the class had a sibling with Down Syndrome. Another teacher related this story:
When I first started the unit, I used Paulo’s Parachute Mission as a read aloud, and we had great discussions on many levels – from the problems Paulo faced … The kids loved this story and almost spoke as if Paulo was their own personal friend … it was really cool. (Survey 4039)
Category 2: Present design challenges that are authentic to engineering practice
2Research indicates that children’s learning is more profound when they engage in realistic disciplinary practices (Roth, 1994; Sawyer, 2006) that include the social and epistemic practices of a discipline and put key concepts into productive use (Duschl, 2008; Duschl & Grandy, 2008; Engle & Conant, 2002; Rosebery, Warren, & Conant, 1992). Students learn concepts and skills through experience as they work and learn in rich contexts that mirror disciplinary problems and practices (Bruner, 2004; Lave & Wenger, 1991; Rogoff, 1990). Recent research has shown that inquiry-based and project-based learning approaches in science lead to comparable or improved outcomes for students compared to more traditional lessons (Anderson, 2002; Blanchard et al., 2010; Geier et al., 2008; Kuhn, 2007; Lehrer & Schauble, 2002; Prince & Felder, 2006; Wilson, Taylor, Kowalski, & Carlson, 2010). Our next five design principles address students’ need for agency and ownership and engineering work that is ‘real.’
Principle 4: Ensure that design challenges are truly open-ended, with more than one ‘correct’ answer
All too often science lessons present tasks where the student’s goal is to arrive at the correct answers. By mid-elementary school, students readily categorize themselves as ‘good at school/science’ or not, based on whether and how quickly they generate the required response.
For teachers, open-ended challenges invite the use of new pedagogical strategies and ways of thinking about learning. For students, particularly those who underperform in a ‘find the correct answer’ system, open-ended challenges are refreshing and can re-engage their efforts. Students more readily make connections with their interests and prior experiences, and they have more reason to listen to and respect the views of others (Järvinen & Twyford, 2000). Open-ended questioning, inquiry, and problem solving also support achievement and positive attitudes for urban minority students, particularly African American boys (Kahle, Meece, & Scantlebury, 2000).
In the real world, engineering problems rarely have one unique solution – much depends on context and constraints. To model this feature of engineering for children, EiE design challenges were intentionally constructed to have multiple solutions. Each design challenge has a set of criteria and constraints, and children evaluate their solutions based on how well they meet these.
For example, in one lesson children are challenged to design a parachute that is ‘mission ready’ – that will fall as slowly as possible in the thin atmosphere of another planet, but also packs into a small space (cargo space is limited on a spacecraft). The two criteria are in tension: larger parachutes tend to fall more slowly, but take up more space. Armed with the results of their experiments testing canopy materials, canopy sizes, and suspension line lengths (see children testing in Figure 3), teams plan, create, and test their parachute designs. Through class sharing, students see that multiple ways of approaching and solving the problem are acceptable.
Principle 5: Value failure for what it teaches
Embracing failure can be a new experience for students. But failure is a necessary and inherent part of the engineering design process. When a design fails, it’s not ‘wrong’ – it invites improved design. EiE activities were designed so children “fail often to succeed sooner” (Nightline, 1999). They invite children to think about engineering as an iterative event – solutions must be redesigned at least once. Initial designs may ‘fail’ to meet the baseline criteria, but these failures help students learn and improve subsequent designs, just like working engineers. Teachers often report that students readily embrace the opportunity to keep honing their solutions, asking if they can keep working on their designs before and after school, during recess, and over holidays. Here are some anecdotes:
“Several students kept bringing in play dough that they made at home. They wanted to improve until they perfected it. When they brought it in, many wanted to share the improvements they made and why they felt it was successful.” (Grade 4 Teacher)
“The majority of my students have continued to test materials and build parachutes during their free time (recess!).” (Grade 3 Teacher)
Working engineers often test their designs to the limit, probing the edge of function to understand under what conditions designs will fail. Testing to failure can be highly engaging for students. One teacher commented, “When we tested our earthquake models, the kids were delighted to be able to shake the daylights out of the structures, and amazed that they remained intact.”
Learning that productive use of failure is a crucial aspect of engineering can be liberating for students – especially for low-achieving students (Meece & Jones, 1996). Instead of worrying about being wrong or looking stupid, students focus on learning from failed attempts as part of an iterative process leading to a feasible solution. A student doesn’t fail; a particular design fails – and hopefully that failure sparks new, improved ideas.
Principle 6: Produce design challenges that can be evaluated with both qualitative and quantitative measures
If a design challenge has multiple solutions, that does not mean every solution addresses design criteria equally well. Children (and adults) may champion their own ideas without adequately considering others’ ideas or conducting objective analyses. EiE design challenges are structured to address this by asking students to collect both qualitative and quantitative data during testing. Students then reflect on the design criteria and assess how well each solution meets them. So instead of a student’s preconceptions, popularity, or perceived ‘smartness’ driving design decisions, objective and impartial data play the key role.
Presenting test results as numbers provides a clear measure for comparison. However, quantification can prove difficult given the tools and instruments typically available in elementary schools. One solution used in some EiE challenges is to ask students to rate a series of variables against a rubric with a numbered scale. The sum of the numbers generated by their observations provides a ‘quantitative’ measure. In other challenges, a simple test can provide a measure. For example, to test the strength of mortar in a stone wall, students use a ‘wrecking ball’ (a golf ball on a string) (http://www.eie.org/eie-curriculum/curriculum-units/sticky-situation-designing-walls). They pull the ball back so the string is at a 10-degree angle and let it drop, then repeat with progressively larger angles, recording the angle at which the wall is destroyed.
Whether measures are quantitative or qualitative, they must be sensibly linked to the goals and context of the design challenge. Although most EiE units engage students in both quantitative and qualitative measurements, the main goal is for students to be able to identify what a successful design is, then develop and improve their own measures. In some EiE units, students develop their own measurement rubrics by considering the desired outcomes of their technology and the ways they might measure them. For example, in the EiE unit Designing a Play Dough Process, (EiE, 2011) http://www.eie.org/eie-curriculum/curriculum-units/work-process-improving-play-dough-process children develop a rubric to measure the quality of play dough, while in Designing Plant Packages (EiE, 2011) http://www.eie.org/eie-curriculum/curriculum-units/thinking-inside-box-designing-plant-packages they develop a rubric to measure plant health. In the EiE unit Evaluating a Landscape presented above, second grade students reasoned about the weight their Tarpuls could carry, using both quantitative measures (how many weights?) and qualitative (whether this was sufficient for a viable design).
Principle 7: Cultivate collaboration and teamwork.
Many popular engineering programs involve contests. But competitive classroom environments that designate winners and losers can differentially discourage participation, especially for girls and underserved minorities (Burke, 2007); many girls and some American minority cultures value interaction and collaboration more highly than competition (Baker & Leary, 1995; Lee, 2003). Given that collaboration is a highly valued skill in both science and engineering and that elementary educators are expected to help their young charges develop the ability to work productively in groups, it makes sense to engage all students in practicing the skills to do this.
Collaboration affords students rich opportunities to develop expertise and identity as valued science and engineering contributors. Learning environments where such interactions are the norm allow students to be valued as contributors in a variety of ways. This reduces the impacts of race and socioeconomic status by allowing students multiple routes to social status instead of sorting and alienating them through hierarchies and competition (Carlone et al., 2011; Olitsky, Flohr, Gardner, & Billups, 2010).
EiE lessons and tasks are designed to foster collaboration and teamwork within and among groups. In almost every activity, children are working either with a partner or with a larger group. The lesson plans make clear that comparing or rank ordering the solutions presented by different student groups is not a goal; rather, each group compares their solution to a set of fixed design criteria and to prior designs the group generated.
EiE teachers frequently mention that learning to work in teams is an essential part of EiE lessons and helps develop this skill. For example, one teacher shared this anecdote:
“Three students who never do anything together – one from Jamaica, one from Sri Lanka, and the third a Bosnian – were teamed up for this project. I marveled at their willingness to accept and test each other’s ideas and analysis without conflict. Their need to test their work and ‘save their frog’ really helped to focus their efforts in a very positive way. To observe them working was a delight; the different perspectives each brought to the task were blended to engineer a very successful membrane.” (Grade 5 Teacher)
Principle 8: Engage students in active, hands-on, inquiry-based engineering
Not surprisingly, children prefer hands-on activities and science experiments over text-based activities; such active learning experiences are still uncommon in many classrooms (Brotman & Moore, 2008) but are integral to every EiE unit. Inquiry-based instruction that emphasizes active student engagement in analyzing and making sense of data is clearly superior to techniques emphasizing passive student learning when it comes to increasing student conceptual understanding (Minner, Levy, & Century, 2010) and inquiry ability (Cuevas, Lee, Hart, & Deaktor, 2005). A variety of studies have shown that two groups in particular benefit from hands-on and inquiry-based learning: girls (Brotman & Moore, 2008; Burkam, Lee, & Smerdon, 1997; Cavallo & Laubach, 2001) and African American students, particularly boys (Kahle et al., 2000). One study found that middle school students using a hands-on engineering design curriculum outperformed students using a traditional science curriculum and students engaged in an inquiry-science unit on an assessment of science reasoning (Silk, Schunn, & Strand Cary, 2009). The evidence that hands-on work promotes engagement, interest, and learning guided the design of EiE. We often get feedback like this comment from one fifth grader: “I liked the way we worked in teams and actually built the circuit instead of just learning about it. I learned much more than I would have if I just read about it.”
Category 3: Scaffold student work
3Students need guidance to learn complex processes and transfer what they have learned to new problems (Mayer, 2004). Instructional methods providing guidance to students who are learning actively have been shown to be consistently superior to pure ‘discovery’ methods where students do not receive guidance (Hmelo-Silver et al., 2007; Mayer, 2004). Scaffolds can include modeling and coaching to make a discipline’s practices and ways of thinking explicit, providing expert hints and explanations, and providing structures and prompts to reduce cognitive load for students engaged in complex tasks (Hmelo-Silver et al., 2007).The following three EiE design principles address scaffolding directly.
Principle 9: Model and make explicit the practices of engineering
Many disciplinary practices and heuristics are specific to engineering. For example, when engineers solve a problem, their work can be generalized into a series of steps called the engineering design process. Similar to the scientific method, this sequence is not absolute or linear; rather it is a tool to guide the work of designers.
Many high school and middle school standards use an engineering design process with 8 or 15 steps (Garmire, 2003; Massachusetts Department of Education, 2006). But elementary teachers insisted that five steps was the maximum number their young charges could remember. This feedback led us to develop a less nuanced (though functionally similar) five-step, cyclical process for younger students. In the context of a design goal, children
- ask questions
- imagine or brainstorm solutions
- choose one design to plan
- create and test a design based on their plan
- analyze and decide how to improve the design
To aid both teachers and children, each step in the EiE engineering design process is accompanied by a series of prompts designed to focus children’s thinking. Teacher materials emphasize that the process is not formulaic and rigid; it is meant as a guide. It can begin with any step, and steps need not be followed in order.
The five-step process is similar to the ‘inquiry framework’ for instructional units described by Cuevas et al (2005), in which students focus on five areas: questioning, planning, implementing, concluding, and reporting. For teachers, Cuevas specified a matrix of different methods of scaffolding appropriate for students with different levels prior experience and skill conducting inquiry. Mirroring our own experience, Cuevas reports that use of an explicit framework and gradual removal of teacher guidance encouraged students to take more initiative and responsibility over time.
Students benefit from the explicit discussion and modeling of other engineering practices. One example is the construction of circuit diagrams in electrical engineering, as in Ms. King’s fifth-grade class:
Ms. K:What’s the difference between the picture of the wire and the diagram of the wire?
Leslie:One is squiggly, the other is straight.
Ms. K:That’s right; in diagrams the wires are always straight, even though they’re not in the real world. So, what symbols do we need to make the classroom diagram?
Jared:A battery.
Ms. K:Come up and get the symbol and put it on the board for us. What else do we need?
Cate:A bulb. (Cate posts the symbol on the board next to the battery.)
Ms. K:If the wires need to be straight, then what will the corners look like?
Tina:Right angles.
Peter:Could it be a triangle?
Ms. K:Do you mean like this? (Ms. K draws a triangle-shaped circuit with parts in it.) It will never be that way. Why do you think so?
Tom:So people don’t wonder why it’s that way.
Ms. K:(Draws a square circuit.) It always has perpendicular lines. Now if I put a bulb here, a battery here – Tina would you draw the wires? (Tina draws another square connecting to the first.)
Ms. K:All right, I’m going to have you practice doing schematic diagrams now.
Brian:Could you have something perpendicular in a different shape?
Ms. K:Draw what you mean on the board, in a different color chalk. (Brian draws a circuit in an L-shape.)
Ms. K:I don’t think it’s wrong, but you want it in the most clear way – like when you do fractions you want the simplest fraction. I’ll give you papers now with a picture of a circuit at the top. Use the symbols and draw a schematic diagram.
In this excerpt, Ms. King works with her students to establish the ‘ground rules’ for making a circuit diagram, including the rule-of-thumb that it should be laid out in the ‘most clear’ way. Circuit diagramming is a form of discourse practice in electrical engineering, and Ms. King’s guided discussion makes explicit the rules of this practice, a form of scaffolding that Nasir, Rosebery, Warren, and Lee (2006) call “making visible the structure of a domain” and that Aikenhead and Jegede (1999) call the teacher’s support of “cultural border crossing.” When we recognize that learning is a cultural process (not only a cognitive process) for all students (Nasir et al., 2006) and that all students need help to become comfortable with the culture of engineering, including its norms and practices, we are able to design learning environments that welcome everyone, without excluding students whose personal cultural worlds are most discordant with the world of engineering.
Principle 10: Assume no previous familiarity with materials, tasks, or terminology
Children enter school with a wide range of experiences and backgrounds that can affect their achievement (Lee, 2003). In an effort to minimize the effects of prior experience on children’s success with engineering tasks, EiE lessons incorporate introductory activities that give children relevant experience with materials and terminology, allowing those who are English Learners or have not previously encountered the materials to engage on a more level playing field.
For example, the project does not assume that children know what a pom-pom is, or how index cards, printer paper, and wax paper are similar or different. Instead a lesson gives children time to interact with the materials, label them, and explore their properties. Teachers remark that such explorations help both children from disadvantaged backgrounds and English Learners. In the following excerpt, Ms. Glick prompts her fourth graders to use varied vocabulary as they explore properties of their material.
Ms. G:Give me some words to describe your play dough.
Jan:Soft.
Child:Sticky.
Joey:Little pieces that are not squishy.
Ms. G:The salt is in there.
Gina:Grains.
Ms. G:Here’s a big word – pliable.
Joey:You can change its shape.
Gina:Movable.
EiE activities are also designed to give students experience with measurement tools including rulers, measuring cups, and weights. They practice cutting, taping, and other construction skills. One elementary science specialist reported, “The kids became experts at measuring, leveling, mixing, etc. They enjoyed all parts of this lesson. It felt more like playing than science. The whole engineering process fitted in with our attempts to make a better play dough.”
Principle 11: Produce activities and lessons that are flexible to the needs and abilities of different kinds of learners
Elementary students differ greatly in their developmental and cognitive abilities. Fortunately, engineering challenges are well suited for differentiated learning. A basic challenge can be made increasingly complex by adding criteria and constraints.
All EiE units offer two levels: basic and advanced. Basic materials, geared toward children in grades 1 and 2, require less reading and writing and offer more pictures than advanced materials, which are geared toward grades 3–5 and include more complicated criteria, constraints, and measures. At both basic and advanced levels, tips for simplifying or expanding the activities and for scaffolding English Learners are provided. Teachers choose the materials that meet their students’ needs.
Many elementary classrooms contain students with a wide range of abilities. One elementary science specialist shared this story:
The class I worked with is by definition a co-taught classroom. Students are placed in the room based on their IEPs. Some are role models. Some children have behavioral challenges. But – these [EiE] lessons really kept them focused and engaged. They anticipated each step, and I feel like it was an outlet for their energy – even reading the stories. Because the unit is activity based, it is a great tool.
A very skilled kindergarten teacher shared this story:
This year I had three children with physician-diagnosed ADHD … They were constantly out of their seats, moving around every 5-10 minutes. Until we started our EiE unit. I was shocked to observe that while they were engaged in the engineering challenges, all of them were focused and on-task for up to 40 minute stretches. Furthermore, although ADHD children often have a difficult time with the writing process … I saw a huge investment and attention to task and detail with the writing components embedded in EiE … It was remarkable! I think the combination of allowing the children to manipulate materials, to engage with them at their own level, to make cross-curricular connections, and to work on a challenge with a goal of finding a solution by trying again and again reached these students as nothing else that year had.
Category 4: Demonstrate that “everyone engineers” and everyone can engineer
4Children can enjoy “doing science’ without wanting to be a scientist; their identity is shaped both by where they come from, in place and in culture, and by their desire to have an effect on the world (Archer et al., 2010). Whether or not children wish to be engineers, we know students are more engaged, interested, and confident when they have both the responsibility and the opportunity to become more competent and to make choices about what forms of competence they wish to ‘specialize’ in.
Our fourth and final category of design principles focuses on students’ self-identification as engineers, or as being good at engineering/understanding the value of engineering.
Principle 12: Cultivate learning environments in which all students’ ideas and contributions have value
When competence is ascribed only to particular individuals, students with lower achievement on standardized measures have less motivation to contribute and participate. Students are more likely to engage when they gain social capital through participation and when their contributions are valued. For example, Roth and Lee (2007) reported that a low-achieving student with learning disabilities became an ‘expert’ when given the opportunity to engage in meaningful practices of environmental science; minority aboriginal students in the same project showed much higher levels of engagement when allowed to choose their mode of participation (Roth & Lee, 2004).
Environments where students are valued for their contributions rather than their test scores are less likely to reinforce socioeconomic inequalities (or ethnic and cultural differences) and more likely to promote broad engagement (Gresalfi, Martin, Hand, & Greeno, 2009; Olitsky et al., 2010). An equitable classroom is one where all students can negotiate and expand the roles they play, where all students share authority, where students are agents with ownership over their learning, and where all students have the opportunity and responsibility to participate with competence (Carlone et al., 2011).
A curriculum that offers equitable, productive engagement for all students must offer a range of means and opportunities for social and cognitive interaction. EiE does this through the careful design of engineering challenges. Students conduct experiments to learn how different materials or procedures perform, and then decide as a group on a strategy for applying what they have learned. Meanwhile engineering challenges are designed to have many possible solutions with competing criteria for success. For example, one group of students may produce a water filter that works quickly, while another may produce one that works slowly but removes more contaminants.
EiE lessons also include participation routines that encourage all students to take responsibility for contributing to the group. For example, students brainstorm individually before sharing their ideas in a group discussion. The combination of complex challenges and varied participation structures allow students myriad opportunities for engaging, contributing, and sharing authority and expertise in the classroom.
Here’s how two teachers reflected on this aspect of the curriculum:
“I got to observe over 90 students being engineers. The best thing for me was how well students got along with other students that they would not usually work with. I saw new friendships build as they worked in teams to make their parachutes. As problems came up, the teams learned that they had to work them out using nice words. Some of the lower students became the leaders of the group when it was hands-on time.” (Grade 3 Teacher)
“It was amazing watching the groups argue, then work cooperatively [to build submersibles] … This class not only learned the concepts of mass, volume, and density, but also were able to apply knowledge into functional learning. They all want to become ocean engineers!” (Grade 2 Teacher)
Principle 13: Foster children’s agency as engineers
An equitable classroom is one where all children can learn, participate, and see how what they are learning is relevant to their lives. When students can develop their identity as someone who is capable of and interested in engineering, they will be more likely to affiliate with the discipline and seek out engineering opportunities in the future. Culture, gender, and socioeconomic status all play into students’ views of themselves and what they think it means to be a ‘science person’ in school (Carlone et al., 2011). Whether they are knowledgeable or struggling, students are more likely to adopt a science (or engineering) identity if they have the opportunity to be active and collaborative producers of knowledge for the classroom community.
EiE lessons deliberately cast students as engineers using language that invites them to engineer solutions to problems. Teachers tell us that children quickly internalize their roles, excitedly proclaiming, “We are going to be mechanical engineers today!” or asking, “When do we get to engineer again?”
When students feel they have agency in their own learning, they are more likely to take initiative. One EiE teacher reported that, after participating in Designing Windmills, two of her students “tracked down the opportunity to visit and tour a [wind] turbine” and one built a model wind turbine. Another teacher told us, “My students really liked the ‘power’ of the decisions they had with this unit. They liked deciding in their groups how they wanted to try something, rather than me telling them what to do!”
Principle 14: Develop challenges that require low-cost, readily available materials
EiE engineering design challenges call for low-cost materials that can be purchased at grocery, craft, or hardware stores – for three reasons. First, children and teachers perceive engineering as more accessible when it calls for ordinary materials. Second, schools often have limited funds for materials. Third, when materials are easy to get, children who find in-school engineering engaging have the opportunity to continue their explorations out of school. Many EiE teachers, including those who work with urban, at-risk youth, report that their pupils do continue to hone their solutions at home, after school or during vacations. One teacher whose class used the Designing Windmills unit reported that two students redesigned their windmill blades at home, resulting in significant improvements in power. Another teacher learned that her students bought out all the flour at the local corner market over spring break as everyone tried improving their play dough design at home. A fourth-grade teacher recounted these two vignettes:
“My bilingual Spanish-English class was working on the Environmental Engineering unit, designing water filters to remove tea, soil, and cornstarch from water. One member of this class was a tall, rather awkward child of African descent from the Dominican Republic. She seemed to lack confidence and had never fully engaged in many academic activities. When we began working on water filtration, she commented that this was a big problem in her home country, and she worked well with her classmates to clean the water during the design challenge. At the end of the unit, she asked to take home a copy of all of her group’s designs. I thought nothing of it and gave her the papers. Several months after we completed the challenge, she came into class and said, “Señora N., lo hice, I did it! I made a filter that gets the water perfectly clear. Could I stay after school and show you my designs?” (I should note that it is really difficult to remove all tea color from water.)
This child had returned home, and because she could readily find the materials for the design challenge (she literally cut up old T-shirts and towels), had continued to work on her filter design for months! I let her show the class her final design. I discussed her interest with her mother, a very supportive woman with limited formal education but a desire to see her daughter triumph in this country. Shortly after, my student told me, ‘For my next challenge my mother has arranged that when I return to the Dominican Republic this summer, I am going to visit a man at a water treatment plant who is working on the desalinization of ocean water for drinking water.’ The EiE challenge had piqued this child’s interest, and she connected it with a problem she knew affected her family’s homeland. This child is now in the gifted and talented program and continues to thrive in math and science at the high school.”
“Last year, I worked with a very challenging student population; many of my students had been exposed to trauma and lacked trust in adults and each other. This was often manifested through a lack of motivation on school assignments, as well as outbursts of anger and defiance. That all changed when my class began working on the EiE chemical engineering unit, in which students design a process to make play dough. My students became so motivated that almost half of the class brought in play dough samples and processes that they had developed at home to try to improve the processes they were working on in class. During class, they would discuss the quality of their samples and try to figure out ways to combine their ideas to come up with the best possible sample. I had to extend the unit for several days as they begged me for ‘more engineering time.’ We had a comparison of wheat flour versus corn flour, as many of my Mexican and Central American students had access to corn flour at home, which brought a cultural dimension to the activities that gave them pride in their identities. By using only materials that even my students of most limited resources had at home, the unit made the concepts accessible to all students. Several students ended the unit saying that they wanted to become engineers as adults, because that was something they knew that they had the ability to do.”
Recommendations
vWell-designed materials are critical for attracting, engaging, and retaining students’ interest in and positive attitudes about engineering. In 2003 the Engineering is Elementary project made a commitment to create curricular materials that would engage all children, including those from underserved and underperforming groups, in problem solving, inquiry, and innovation. Drawing on related research and our own experiences, we articulated design principles and applied them in the development of 20 elementary curriculum units.
We invite other curriculum designers to use the 14 inclusive principles articulated here as a starting point for a conversation about resource design. We encourage others involved in K-12 engineering programming and resource development to carefully consider other ways to actively reach out to underrepresented populations – for unless we do, the status quo will continue.
Obviously, more research is needed, both about elementary-level engineering and about how to develop materials that engage all students in engineering. We need to understand more about how to design curricular materials so they scaffold student learning. We are intrigued by the possibilities that digital technologies might play in supporting various learning styles in engineering activity. We need more information about how children’s understandings of engineering principles and habits of mind develop so we can reflect these in our curricular resources. EiE is currently conducting research that measures the impact of our curricular design principles on students, particularly children from underrepresented groups. We welcome the application, adoption, and modification of our principles by others, and we will continue to revise and refine these principles as we learn more.
Acknowledgement
viThis article is a condensed version of Cunningham C. M. & Lachapelle, C. P. (2014) and is presented here by kind permission of the publisher, Purdue University Press.
[1] Our work has primarily focused on the United States so the literature we looked to was based largely in the U.S.A. and the field testing we conducted was exclusively in this country.
References
viiAikenhead, G. S., & Jegede, O. J. (1999). Cross-cultural science education: A cognitive explanation of a cultural phenomenon. Journal of Research in Science Teaching, 36(3), 269–287. http://dx.doi.org/10.1002/(SICI)1098-2736(199903)36:3<269::AID-TEA3>3.0.CO;2-T
Anderson, R. (2002). Reforming science teaching: What research says about inquiry. Journal of Science Teacher Education, 13(1), 1–12. http://dx.doi.org/10.1023/A:1015171124982
Archer, L., DeWitt, J., Osborne, J., Dillon, J., Willis, B., & Wong, B. (2010). “Doing” science versus “being” a scientist: Examining 10/11-year-old schoolchildren's constructions of science through the lens of identity. Science Education, 94(4), 617–639. http://dx.doi.org/10.1002/sce.20399
Baker, D., & Leary, R. (1995). Letting girls speak out about science. Journal of Research in Science Teaching, 32(1), 3–27. http://dx.doi.org/10.1002/tea.3660320104
Blanchard, M. R., Southerland, S. A., Osborn, J. W., Sampson, V. D., Annetta, L. A., & Granger, E. M. (2010). Is inquiry possible in light of accountability?: A quantitative comparison of the relative effectiveness of guided inquiry and traditional verification laboratory instruction. Science Education, 94(4), 577–616. http://dx.doi.org/10.1002/sce.20390
Blumenfeld, P., Soloway, E., Marx, R., Krajcik, J., Guzdial, M., & Palincsar, A. (1991). Motivating project-based learning: Sustaining the doing, supporting the learning. Educational Psychologist, 26(3) , 369–398. http://dx.doi.org/10.1207/s15326985ep2603&4_8
Brotman, J. S., & Moore, F. M. (2008). Girls and science: A review of four themes in the science education literature. Journal of Research in Science Teaching, 45(9), 971–1002.http://dx.doi.org/10.1002/tea.20241
Bruner, J. S. (2004). A short history of psychological theories of learning. Daedalus, 133(1), 13–20. http://dx.doi.org/10.1162/001152604772746657
Buccheri, G., Gurber, N. A., & Bruhwiler, C. (2011). The impact of gender on interest in science topics and the choice of scientific and technical vocations. International Journal of Science Education, 33, 159–178. http://dx.doi.org/10.1080/09500693.2010.518643
Burkam, D. T., Lee, V. E., & Smerdon, B. A. (1997). Gender and science learning early in high school: Subject matter and laboratory experiences. American Educational Research Journal, 34(2), 297–331. http://dx.doi.org/10.3102/00028312034002297
Burke, R. J. (2007). Women and minorities in STEM: A primer. In R. J. Burke & M. C. Mattis (Eds.), Women and Minorities in Science, Technology, Engineering and Mathematics (pp. 3–27). Northampton, MA: Edward Elgar Publishing. http://dx.doi.org/10.4337/9781847206879.00008
Burke, R. J., & Mattis, M. C. (Eds.). (2007). Women and minorities in science, technology, engineering and mathematics: Upping the numbers. Northampton, MA: Edward Elgar Publishing. http://dx.doi.org/10.4337/9781847206879
Buxton, C. A. (2010). Social problem solving through science: An approach to critical, place-based science teaching and learning. Equity & Excellence in Education, 43(1), 120 - 135. http://dx.doi.org/10.1080/10665680903408932
Catsambis, S. (1995). Gender, race, ethnicity, and science education in the middle grades. Journal of Research in Science Teaching, 32(3), 243–257. http://dx.doi.org/10.1002/tea.3660320305
Cavallo, A. M. L., & Laubach, T. A. (2001). Students’ science perceptions and enrollment decisions in differing learning cycle classrooms. Journal of Research in Science Teaching, 38(9), 1029–1062. http://dx.doi.org/10.1002/tea.1046
Cuevas, P., Lee, O., Hart, J., & Deaktor, R. (2005). Improving science inquiry with elementary students of diverse backgrounds. Journal of Research in Science Teaching, 42(3), 337–357. http://dx.doi.org/10.1002/tea.20053
Dawson, C. (2000). Upper primary boys’ and girls’ interests in science: Have they changed since 1980? International Journal of Science Education, 22(6), 557 - 570. http://dx.doi.org/10.1080/095006900289660
Drechsel, B., Carstensen, C., & Prenzel, M. (2011). The role of content and context in PISA interest scales: A study of the embedded interest items in the PISA 2006 science assessment. International Journal of Science Education, 33(1), 73–95. http://dx.doi.org/10.1080/09500693.2010.518646
Duschl, R. A. (2008). Science education in three-part harmony: Balancing conceptual, epistemic, and social learning goals. Review of Research in Education, 32(1), 268–291. http://dx.doi.org/10.3102/0091732X07309371
Engineering is Elementary (EiE). (2011). Engineering curriculum for grades 1–5. Boston, MA: Museum of Science. http://www.eie.org/eie-curriculum
Engle, R. A., & Conant, F. R. (2002). Guiding principles for fostering productive disciplinary engagement: Explaining an emergent argument in a community of learners classroom. Cognition and Instruction, 20, 399–483. http://dx.doi.org/10.1207/S1532690XCI2004_1
Geier, R., Blumenfeld, P. C., Marx, R. W., Krajcik, J. S., Fishman, B., Soloway, E., & Clay-Chambers, J. (2008). Standardized test outcomes for students engaged in inquiry based science curricula in the context of urban reform. Journal of Research in Science Teaching, 45(8), 922–939. http://dx.doi.org/10.1002/tea.20248
Gresalfi, M., Martin, T., Hand, V., & Greeno, J. (2009). Constructing competence: An analysis of student participation in the activity systems of mathematics classrooms. Educational Studies in Mathematics, 70(1), 49–70. http://dx.doi.org/10.1007/s10649-008-9141-5
Halpern, D. F., Aronson, J., Reimer, N., Simpkins, S., Star, J. R., & Wentzel, K. (2007). Encouraging girls in math and science. NCER 2007–2003. Washington, DC: National Center for Education Research, Institute of Education Science, U.S. Department of Education. http://nrs.harvard.edu/urn-3:HUL.InstRepos:4889482
Järvinen, E.M., & Twyford, J. (2000). The influences of socio-cultural interaction upon children’s thinking and actions in prescribed and open-ended problem solving situations (An investigation involving design and technology lessons in English and Finnish primary schools). International Journal of Technology and Design Education, 10(1), 21–41. http://dx.doi.org/10.1023/A:1008996305565
Jones, M. G., Howe, A., & Rua, M. J. (2000). Gender differences in students' experiences, interests, and attitudes toward science and scientists. Science Education, 84(2), 180–192. http://dx.doi.org/10.1002/(SICI)1098-237X(200003)84:2<180::AID-SCE3>3.0.CO;2-X
Kahle, J. B., Meece, J., & Scantlebury, K. (2000). Urban African-American middle school science students: Does standards-based teaching make a difference? Journal of Research in Science Teaching, 37(9), 1019–1041. http://dx.doi.org/10.1002/1098-2736(200011)37:93.0.CO;2-J
Kang, H., & Lundeberg, M. A. (2010). Participation in science practices while working in a multimedia case-based environment. Journal of Research in Science Teaching, 47(9), 1116–1136. http://dx.doi.org/10.1002/tea.20371
Klassen, S. (2007). The application of historical narrative in science learning: The atlantic cable story. Science & Education, 16(3), 335–352. http://dx.doi.org/10.1007/s11191-006-9026-x
Klassen, S. (2009). The construction and analysis of a science story: A proposed methodology. Science & Education, 18(3), 401–423. http://dx.doi.org/10.1007/s11191-008-9141-y
Koul, R., & Dana, T. M. (1997). Contextualized science for teaching science and technology. Interchange, 28(2), 121–144. http://dx.doi.org/10.1023/A:1007348821292
Kuhn, D. (2007). Reasoning about multiple variables: Control of variables is not the only challenge. Science Education, 91(5), 710–726. http://dx.doi.org/10.1002/sce.20214
Lave, J., & Wenger, E. (1991). Situated learning : legitimate peripheral participation. New York: Cambridge University Press. http://dx.doi.org/10.1017/CBO9780511815355
Lee, O. (2003). Equity for linguistically and culturally diverse students in science education: A research agenda. Teachers College Record, 105(3), 465–489. . http://www.tcrecord.org/Issue.asp?volyear=2003&number=3&amo;volume=105
Lieberman, G. A., & Hoody, L. L. (1998). Closing the achievement gap: Using the environment as an integrating context for learning. Results of a nationwide study. http://www.seer.org/pages/GAP.html
Martin, B. E., & Brouwer, W. (1991). The sharing of personal science and the narrative element in science education. Science Education, 75(6), 707–722. http://dx.doi.org/10.1002/sce.3730750610
Meece, J. L., & Jones, M. G. (1996). Gender differences in motivation and strategy use in science: Are girls rote learners? Journal of Research in Science Teaching, 33(4), 393–406. http://dx.doi.org/10.1002/(SICI)1098-2736(199604)33:4<393::AID-TEA3>3.0.CO;2-N
Miller, P. H., Blessing, J. S., & Schwartz, S. (2006). Gender differences in high-school students’ views about science. International Journal of Science Education 28(4), 363–381. http://dx.doi.org/10.1080/09500690500277664
Minner, D. D., Levy, A. J., & Century, J. (2010). Inquiry-based science instruction – what is it and does it matter? Results from a research synthesis years 1984 to 2002. Journal of Research in Science Teaching, 47(4), 474–496. http://dx.doi.org/10.1002/tea.20347
Olitsky, S., Flohr, L. L., Gardner, J., & Billups, M. (2010). Coherence, contradiction, and the development of school science identities. Journal of Research in Science Teaching, 47(10), 1209–1228. http://dx.doi.org/10.1002/tea.20389.
Prince, M., & Felder, R. (2006). Inductive teaching and learning methods: Definitions, comparisons, and research bases. Journal of Engineering Education, 95, 123–138. http://dx.doi.org/10.1002/j.2168-9830.2006.tb00884.x
Reid, N., & Skryabina, E. A. (2003). Gender and physics. International Journal of Science Education, 25(4), 509 - 536. http://dx.doi.org/10.1080/0950069022000017270
Rosebery, A. S., Warren, B., & Conant, F. R. (1992). Appropriating scientific discourse: Findings from language minority classrooms. [CPL]. Journal of the Learning Sciences, 2(1), 61–94. http://dx.doi.org/10.1207/s15327809jls0201_2
Roth, W. M. (1994). Experimenting in a Constructivist High-School Physics Laboratory. Journal of Research in Science Teaching, 31(2), 197–22. http://dx.doi.org/10.1002/tea.3660310209
Roth, W. M., & Lee, S. (2004). Science Education as/for Participation in the Community. Science Education, 88(2), 263–29. http://dx.doi.org/10.1002/sce.10113
Roth, W. M., & Lee, Y.-J. (2007). "Vygotsky's neglected legacy": Cultural-historical activity theory. Review of Educational Research, 77(2), 186–232. http://dx.doi.org/10.3102/0034654306298273
Silk, E. M., Schunn, C. D., & Strand Cary, M. (2009). The impact of an engineering design curriculum on science reasoning in an urban setting. Journal of Science Education and Technology, 18(3), 209–223. http://dx.doi.org/10.1007/s10956-009-9144-8
Stinner, A. (1996). Providing a contextual base and a theoretical structure to guide the teaching of science from early years to senior years. Science & Education, 5(3), 247–266. http://dx.doi.org/10.1007/BF00414315
Weis, A. M., & Banilower, E. R. (2010). Engineering is Elementary: Impact on historically-underrepresented students’ survey results. Chapel Hill, NC: Horizon Research, Inc.
About the Authors
viiiDr. Christine Cunningham is an educational researcher who works to make engineering and science more relevant, accessible, and understandable, especially for underserved and underrepresented populations. A vice president at the Museum of Science, Boston since 2003, she founded and directs Engineering is Elementary, a groundbreaking project that integrates engineering concepts into elementary curriculum and teacher professional development. As of February 2016, EiE has served 10 million children nationwide and 110,000 educators. Cunningham is a Fellow of the American Society for Engineering Education and was awarded the 2014 International Society for Design and Development in Education Prize and the 2015 IEEE Pre-University Educator Award.
Dr. Cathy Lachapelle leads the Engineering is Elementary team responsible for assessment and evaluation of our curricula. This includes the design and field-testing of assessment instruments and research on how children use EiE materials. Cathy is particularly interested in how collaborative interaction and scaffolded experiences with disciplinary practices help children learn science, math, and engineering.